There are two primary reasons for grounding devices, cables, equipment, and systems. The first reason is to prevent shock and fire hazards in the event that an equipment frame or housing develops a high voltage due to lightning or an accidental breakdown of wiring or components. The second reason is to reduce EMI effects resulting from electromagnetic fields, common impedance, or other forms of interference coupling.

Historically, grounding requirements arose from the need to provide protection from electrical faults, lightning, and industrially-generated static electricity. Because most power-fault and lightning control relies on a low-impedance path to earth, all major components of an electrical power generation and transmission system were earth grounded to provide the required low-impedance path. As a result, strong emphasis was placed on earth grounding of electrical equipment, and the overall philosophy was “ground, ground, ground” without regard to other problems, such as EMI, that may be created by this approach.
When electronic equipment was introduced, grounding problems became more evident. These problems resulted from the fact that the circuit and equipment grounds often provided the mechanism for undesired EMI coupling. Also, with electronic systems, the ground may simultaneously perform two or more functions, and these multiple functions may be in conflict either in terms of operational requirements or in terms of implementation techniques. For example, as illustrated in Figure 1, the ground network for an electronic equipment may be used as a signal return, provide safety, provide EMI control, and also perform as part of an antenna system.
Therefore, in order to avoid creating EMI problems, it is essential to recognize that an effective grounding system, like any other portion of an equipment or system, must be carefully designed and implemented. Grounding is a system problem and in order for a grounding arrangement to perform well it must be well conceived and accurately designed and implemented. The grounding configurations must be weighed with regard to dimensions and frequency, just like any functional circuit.
The objective of this chapter is to help engineers, designers, and technicians to optimize the functionality and reliability of their equipment by providing an orderly systems approach to grounding. Such an approach is highly preferable to the empirical and sometimes contradictory approaches that are often employed.
Characteristics of Grounding Systems
Ideally, a ground system should provide a zero-impedance path to all signals for which it serves as a reference. If this were the situation, signal currents from different circuits or equipment that are connected to the ground could return to their respective sources without creating unwanted coupling between the circuits or equipment. Many interference problems occur because designers treat the ground as ideal and fail to give proper attention to the actual characteristics of the grounding system. One of the primary reasons that designers treat the ground system as ideal is that this assumption is often valid from the standpoint of the circuit or equipment design parameters (i.e., the impedance at power or signal frequencies is small and has little or no impact on circuit or equipment performance). However, the non-ideal properties of the ground must be recognized if EMI problems are to be avoided.
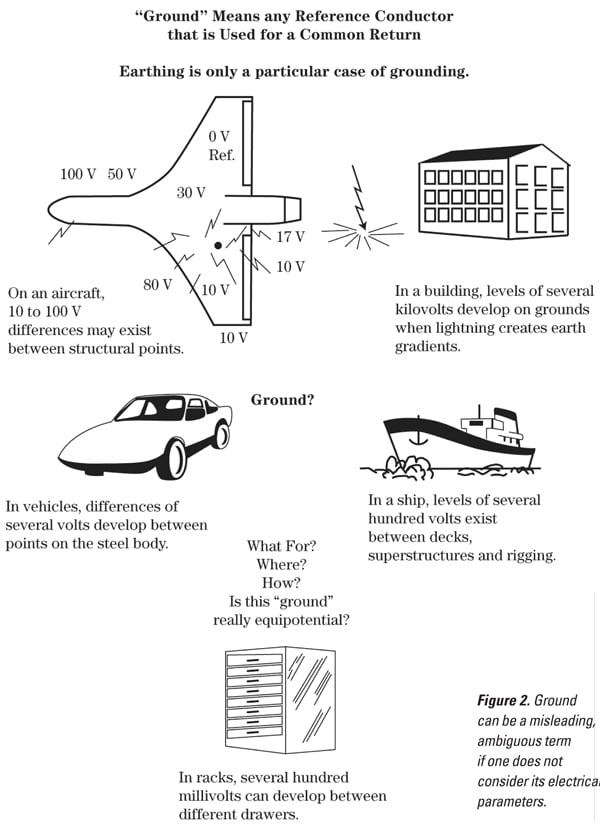
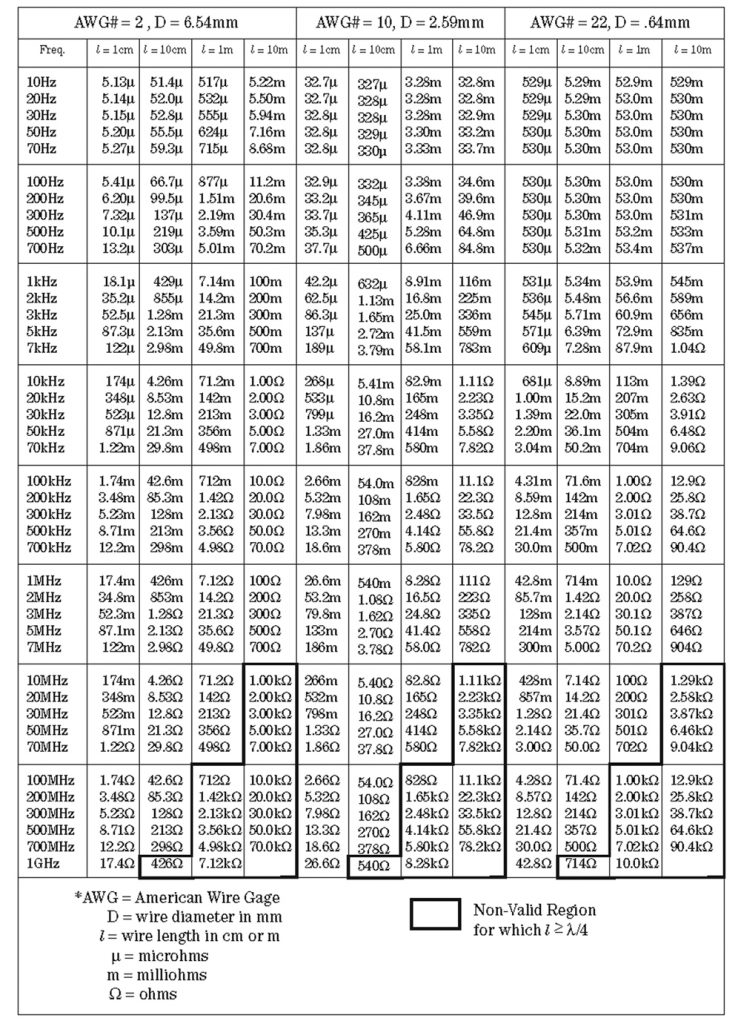
Impedance Characteristics
Every element (conductor) of a grounding system, whether it be for power grounding, signal grounding, or lightning protection, has properties of resistance, capacitance, and inductance. Shields and drain wires of signal cables, the green wire power safety ground, lightning down conductors, transformer vault buses, structural steel members — all conductors have these properties. The resistance property is exhibited by all metals. The resistance of a ground path conductor is a function of the material, its length, and its cross-sectional area. The capacitance associated with a ground conductor is determined by its geometric shape, its proximity to other conductors, and the nature of the intervening dielectric. The inductance is a function of its size, geometry, length, and, to a limited extent, the relative permeability of the metal. The impedance of the grounding system is a function of the resistance, inductance, capacitance, and frequency.
Because the inductance properties of a conductor decrease with width and increase with length, it is frequently recommended that a length-to-width ratio of 5:1 be used for grounding straps. This 5:1 length-to-width ratio provides a reactance that is approximately 45 percent of that of a straight circular wire.
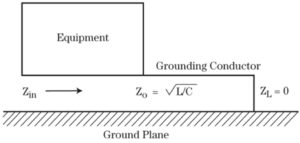
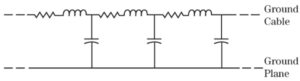
The impedance of straight circular wires is provided as a function of frequency in Table 5.1 for several wire gauges and lengths. Typical ground plane impedances are provided in Table 5.2 for comparison. Note that for typical length wires, ground plane impedances are several orders of magnitude less than those of a circular wire. Also note that the impedance of both circular wires and ground planes increase with increasing frequency and become quite significant at higher frequencies.
A commonly encountered situation is that of a ground cable (power or signal) running along in the proximity of a ground plane. This situation is illustrated in Figure 3 for equipment grounding. Figure 4 illustrates a representative circuit of this simple ground path. The effects of the resistive elements of the circuit will predominate at very low frequencies.
The relative influence of the reactive elements will increase at increasing frequencies. At some frequency, the magnitude of the inductive reactance (jωL) equals the magnitude of the capacitive reactance (1/jωC), and the circuit becomes resonant. The frequency of the primary (or first) resonance can be determined from:
where L is the total cable inductance, and C is the net capacitance between the cable and the ground plane. At resonance, the impedance presented by the grounding path will either be high or low, depending on whether it is parallel or series resonant, respectively. At parallel resonance, the impedance seen looking into one end of the cable will be much higher than expected from R + jωL. (For good conductors, e.g., copper and aluminum, R « ωL; thus, jωL generally provides an accurate estimate of the impedance of a ground conductor at frequencies above a few hundred hertz). At parallel resonance:
where Q, the quality factor, is defined as:
where R(ac) is the cable resistance at the frequency of resonance. Then:
Above the primary resonance, subsequent resonances (both parallel and series) will occur between the various possible combinations of inductances and capacitances (including parasitics) in the path.Series resonances in the grounding circuit will also occur between the inductances of wire segments and one or more of the shunt capacitances. The impedance (Zs) of a series resonant path is:
Therefore,
The series resonant impedance is thus determined by, and is equal to, the series ac resistance of the particular inductance and capacitance in resonance. (At the higher ordered resonances, where the resonant frequency is established by wire segments and not the total path, the series impedance of the path to ground may be less than predicted from a consideration of the entire ground conductor length).
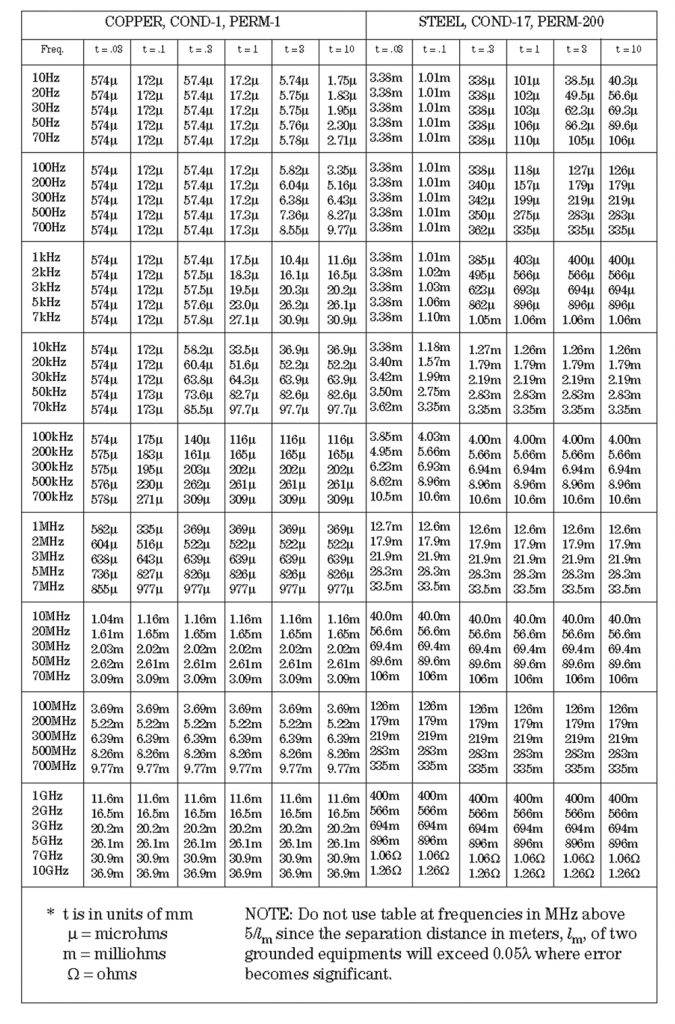
An understanding of the high-frequency behavior of a grounding conductor is simplified by viewing it as a transmission line. If the ground path is considered uniform along its run, the voltages and currents along the line can be described as a function of time and distance. If the resistance elements in Figure 4 are small relative to the inductances and capacitances, the grounding path has a characteristic impedance, Z0, equal to √L/C where L and C are the per-unit length values of inductance and capacitance.
The situation illustrated in Figure 3 is of particular interest in equipment grounding. The input impedance of the grounding path, i.e., the impedance to ground seen by the equipment case, is:
where, ß=ω√LC= the phase constant for the transmission line
x = the length of the path from the box to the short
where ßx is less than π/2 radians, i.e., when the electrical path length is less than a quarter wavelength (λ/4), the input impedance of the short-circuited line is inductive with a value ranging from 0 (ßx= 0) to ∞(ßx= p/2 radians). As ßx= increases beyond π/2 radians in value, the impedance of the grounding path cycles alternately between its open- and short-circuit values.
Thus, from the vantage point of the device or component that is grounded, the impedance is analogous to that offered by a short-circuited transmission line. Where ßx= π/2, the impedance offered by the ground conductor behaves like a lossless parallel LC resonant circuit. Just below resonance, the impedance is inductive; just above resonance, it is capacitive; while at resonance, the impedance is real and quite high (infinite in the perfectly lossless case). Resonance occurs at values of x equal to integer multiples of quarter wavelengths, such as a half wavelength, three-quarter wavelength, etc.
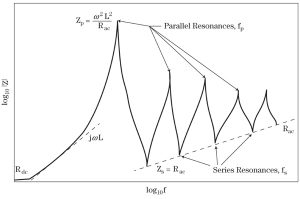
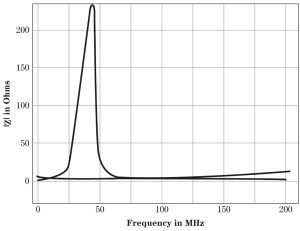
Typical ground networks are complex circuits of Rs, Ls, and Cs with frequency-dependent properties including both parallel and series resonances. These resonances are important to the performance of a ground network. Resonance effects in a grounding path are illustrated in Figure 5. The relative effectiveness of a grounding conductor as a function of frequency is directly related to its impedance behavior (Figure 6).
It is evident from Figures 5 and 6 that, for maximum efficiency, ground conductor lengths should be a small portion of the wavelength at the frequency of the signal of concern. The most effective performance is obtained at frequencies well below the first resonance.
Antenna Characteristics
Antenna effects are also related to circuit resonance behavior. Ground conductors will act as antennas to radiate or pick up potential interference energy, depending on their lengths relative to a wavelength, i.e., their efficiency. This fact permits a wavelength-to-physical-length ratio to be derived for ground conductors.
The efficiency of a conductor as an antenna is related to its radiation resistance. Radiation resistance is a direct measure of the energy radiated from the antenna. A good measure of performance for a wire is a quarter-wave monopole, which has a radiation resistance of 36.5 W. An antenna that transmits or receives 10 percent or less than a monopole can be considered to be inefficient. To be effective, a ground wire should be an inefficient antenna. A convenient criterion for a poor antenna, i.e., a good ground wire, is that its length be l/10 or less. Thus, a recommended goal in the design of an effective grounding system is to maintain ground wires exposed to potentially interfering signals at lengths less than 1/10 of a wavelength of the interfering signal.
GROUND-RELATED INTERFERENCE
Interference is any extraneous electrical or electromagnetic disturbance that tends to disrupt the reception of desired signals or produces undesirable responses in a circuit or system. Interference can be produced by both natural and man-made sources, either external or internal to the circuit. The correct operation of complex electronic equipment and facilities is inherently dependent upon the frequencies and amplitudes of both the signals utilized in the system and the potential interference emissions that are present. If the frequency of an undesired signal is within the operating frequency range of a circuit, the circuit may respond to the undesired signal (it may even happen out of band). The severity of the interference is a function of the amplitude and frequency of the undesired signal relative to that of the desired signal at the point of detection.
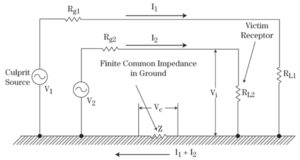
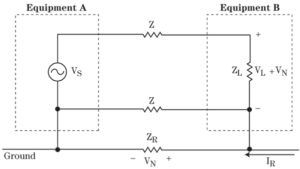
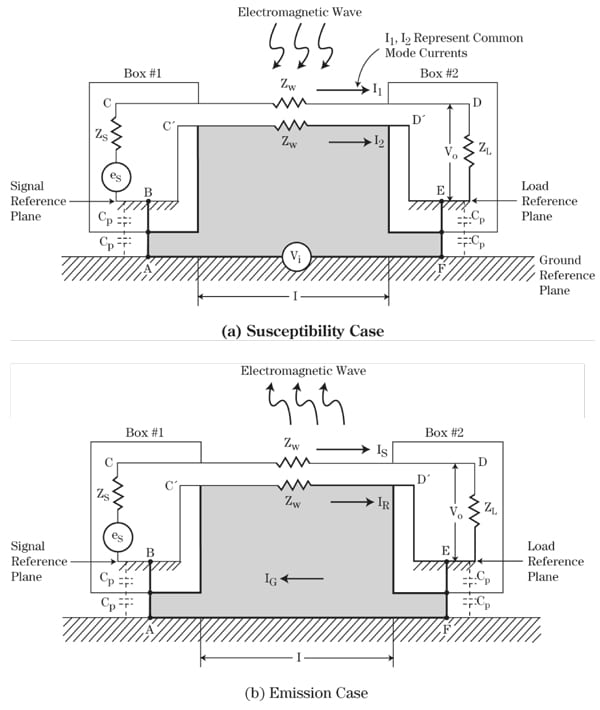
Ground-related interference often involves one of two basic coupling mechanisms. The first mechanism results from the fact that the signal circuits of electronic equipment share the ground with other circuits or equipment. This mechanism is called common-ground impedance coupling. Any shared impedance can provide a mechanism for interference coupling. Figure 7 illustrates the mechanism by which interference is coupled between culprit and victim circuits via the common-ground impedance. In this case, the interference current, I, flowing through the common-ground impedance, Z, will produce an interfering signal voltage, Vc, in the victim circuit. It should be emphasized that the interference current flowing in the common impedance may be either a current that is related to the normal operation of the culprit circuit or an intermittent current that occurs due to abnormal events (lightning, power faults, load changes, power line transients, etc.).
Even if the equipment pairs do not use the signal ground as the signal return, the signal ground can still be the cause of coupling between them. Figure 8 illustrates the effect of a stray current, IR, flowing in the signal ground. The current IR may be the result of the direct coupling of another equipment pair to the signal ground. It may be the result of external coupling to the signal ground, or induced in the ground by an incident field. In either case, IR produces a voltage VN in the ground impedance ZR. This voltage produces a current in the interconnecting loop, which in turn develops a voltage across ZL in Equipment B. Thus, it is evident that interference can conductively couple through the signal ground to all circuits and equipment connected across the non-zero impedance elements of that ground.
The second EMI coupling mechanism involving ground is a radiation mechanism whereby the ground loop, as shown in Figure 9, acts as a receiving or transmitting antenna. For this EMI coupling mechanism, the characteristics of the ground (resistance or impedance) do not play an important role, because the induced EMI voltage (for the susceptibility case) or the emitted EMI field (for the emission case) is mainly a function of the EMI driving function (field strength, voltage, or current), the geometry and dimensions of the ground loop, and the frequency of the EMI signal. It should be noted that both the conducted and radiated EMI coupling mechanisms identified above involve a “ground loop.” However, it should be recognized that ground loop EMI problems can exist without a physical connection to ground. In particular, at RF frequencies, distributed capacitance to ground can create a ground loop condition even though circuits or equipment are floated with respect to ground.
Also, it should be noted that, for both of the EMI coupling mechanisms involving the ground loop, the EMI currents in the signal lead and the return are flowing in the same direction. This EMI condition (where the currents in the signal lead and the return are in phase) is referred to as common-mode EMI. The EMI control techniques that will be effective for ground loop problems are those that either reduce the coupling of EMI into the ground loop or provide suppression of the common-mode EMI that is coupled into the ground loop.
Circuit, Equipment, and System Grounding
In the previous section, EMI coupling mechanisms resulting from circuit, equipment, and system grounding were identified and discussed.At this point, it should be obvious that grounding is very important from the standpoint of minimizing and controlling EMI. However, grounding is one of the least understood and most significant culprits in many system-level EMI problems. The grounding scheme of a system must perform the following functions:
- Analog, low-level, and low-frequency circuits must have noise-free dedicated returns. Due to the low frequencies involved, wires are generally used (more or less dictating a single-point or star ground system).
- Analog high-frequency circuits {radio, video, etc.} must have low impedance, noise-free return circuits, generally in form of planes or coaxial cables.
- Returns of logic circuits, especially high-speed logic, must have low impedances over the whole bandwidth (dictated by the fastest rise times), since power and signal returns share the same paths.
- Returns of powerful loads (solenoids, motors, lamps, etc.) should be distinct from any of the above, even though they may end up in the same terminal of the power supply regulator.
- Return paths to chassis of cable shields, transformer shields, filters, etc. must not interfere with functional returns.
- When the electrical reference is distinct from the chassis ground, provision and accessibility must exist to connect and disconnect one from the other.
- More generally, for signals that communicate within the equipment or between parts of a system, the grounding scheme must provide a common reference with minimum ground shift (unless these links are balanced, optically isolated, etc.). Minimum ground shift means that the common-mode voltage must stay below the sensitivity threshold of the most susceptible device in the link.
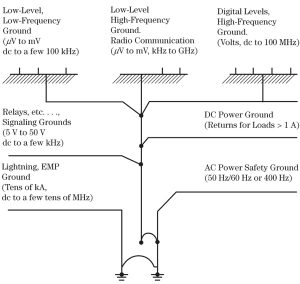
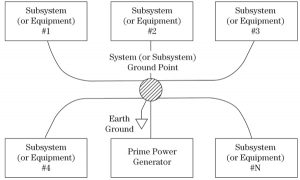
All the above constraints can be accommodated if their functional returns and protective grounds are integrated into a grounding system hierarchy as shown in Figure 10. The application of this concept is the subject of the following discussion.
Modern electronic systems seldom have only one ground. To mitigate interference, such as due to common-mode impedance coupling, as many separate grounds as possible are used. Separate grounds in each subsystem for structural grounds, signal grounds, shield grounds, and primary and secondary power grounds are desirable if economically and logistically practical. These individual grounds from each subsystem are finally connected by the shortest route back to the system ground point, where they form an overall system potential reference. This method is known as a single-point ground and is illustrated in Figure 11.
Single-Point Grounding Scheme
The single-point or star type of grounding scheme shown in the figure avoids problems of common-mode impedance coupling discussed in the previous section. The only common path is in the earth ground (for earth-based structures), but this usually consists of a substantial conductor of very-low impedance. Thus, as long as no or low ground currents flow in any low-impedance common paths, all subsystems or equipments are maintained at essentially the same reference potential.
The problem of implementing the above single-point grounding scheme comes about when (1) interconnecting cables are used, especially ones having cable shields that have lengths on the order of 1/20 of a wavelength or greater, and (2) parasitic capacitance exists between subsystem or equipment housings or between subsystems and the grounds of other subsystems. This situation is illustrated in Figure 12.

Here, cable shields connect some of the subsystems together so that more than one grounding path from a particular subsystem to the ground point exists. Unless precautions are taken, common-impedance ground currents could flow. At high frequencies, the parasitic capacitive reactance represents low-impedance paths, and the bond inductance of a subsystem-to-ground point results in higher impedances. Thus, again, common-mode currents may flow or unequal potentials may develop between subsystems.
Multipoint Grounding Scheme
Rather than have an uncontrolled situation as shown in Figure 12, the other grounding alternative is multipoint grounding as illustrated in Figure 13. For the example shown in Figure 13, each equipment or subsystem is bonded as directly as possible to a common low-impedance ground plane to form a homogeneous, low-impedance path. Thus, common-mode currents and other EMI problems will be minimized. The ground plane then is earthed for safety purposes.

Selection of a Grounding Scheme
The facts are that a single-point grounding scheme operates better at low frequencies, and a multipoint ground behaves best at high frequencies. If the overall system, for example, is a network of audio equipment, with many low-level sensors and control circuits behaving as broadband transient noise sources, then the high-frequency performance is irrelevant, since no receptor responds above audio frequency.
For this situation, a single-point ground would be effective. Conversely, if the overall system were a receiver complex of 30 to 1,000 MHz tuners, amplifiers, and displays, then low-level, low-frequency performance is irrelevant. Here, multipoint grounding applies, and interconnecting coaxial cables should be used.
The above comparison of audio versus VHF/UHF systems makes clear the selection of the correct approach. The problem then narrows down to one of defining where low- and high-frequency crossover exists for any given subsystem or equipment. The answer here in part involves the highest significant operating frequency of low-level circuits relative to the physical distance between the farthest located equipments.The determination of the crossover frequency region involves consideration of (1) magnetic versus electric field coupling problems and (2) ground-plane impedance problems due to separation. Hybrid single and multipoint grounding systems are often the best approach for crossover region applications.
When printed circuits and ICs are used, network proximity is considerably closer. Thus, multipoint grounding is more economical and practical to produce per card, wafer, or chip. Interconnection of these components through wafer risers, motherboards, etc. should use a grounding scheme following the illustrations of previous paragraphs.
This will likely still represent a multipoint or hybrid grounding approach in which any single-point grounding (for hybrid grounds), if used, would be to avoid low-frequency ground current loops and/or common-mode impedance coupling.In summary, many system-level EMI problems can be avoided by paying careful attention to the grounding scheme used. Common-mode, common-ground impedance problems may be reduced by application of one or more of the following techniques.
- Eliminate common impedance by using a single point ground (Figure 11) if possible. This configuration is usually optimal for power frequencies and signal frequencies below 300 kHz.
- Separate and isolate grounds on the basis of signal type, level, and frequency as illustrated in Figure 10.
- Minimize ground impedance as illustrated in Figure 14 by using ground bus, ground plane, or ground grid.
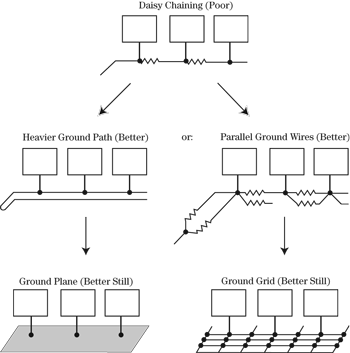
- Float circuits or equipments if practical from a safety standpoint as illustrated in Figure 15. The effectiveness of floating circuits or equipments depends on their physical isolation from other conductors. In large facilities, it is difficult to achieve a floating system.

- Use an inductor or capacitor in the ground connection to provide high- or low-frequency isolation, respectively, as illustrated in Figures 16 and 17.


- Use filters or ferrites in ground loops to limit common-mode currents or provide a common-mode voltage drop.
- Use a common-mode choke as illustrated in Figure 18 or a common mode isolation transformer as illustrated in Figure 19 to suppress ground-loop EMI. These devices may provide on the order of 60 dB of common-mode rejection at frequencies up to several hundred kilohertz.

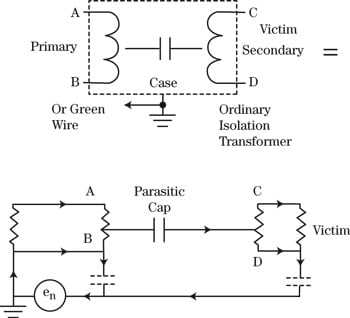
- Use optical isolators and/or fiber optics to block common-mode EMI effects as illustrated in Figure 20. Optical isolators provide a high degree of common-mode rejection at frequencies up to and including the HF band (i.e., 3 to 30 MHz). Optical isolators are usually limited to digital applications (they are not applicable to low-level analog circuits).
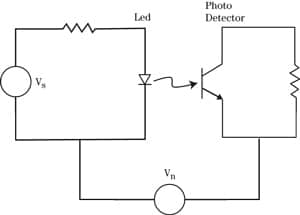
- Use balanced circuits to minimize effects of common-mode EMI in the ground loop as illustrated in Figure 21. With a perfectly balanced circuit, the currents flowing in the two parts of the circuit will produce equal and opposite voltages across the load, so the resulting voltage across the load is zero. Balanced circuits can provide significant (greater than 20 dB) common-mode reduction for low-frequency conditions. However, at higher frequencies (above 30 MHz), other effects start to predominate, and the effectiveness of balanced circuits diminishes.

Common-mode radiated EMI effects resulting from emissions that are radiated or picked up by a ground loop may be reduced by the application of one or more of the following techniques:
- Minimize the common-mode ground loop area by routing interconnecting wires or cable close to the ground.
- Reduce the common-mode ground loop currents by floating circuits or equipments; using optical isolators; or inserting common-mode filters, chokes, or isolation transformers.
- Use balanced circuits or balanced drivers and receivers.
Ground System Configurations
The ground system for a collection of circuits within a system or facility can assume any one of several different configurations. Each of these configurations tends to be optimal under certain conditions and may contribute to EMI problems under other conditions. In general, the ground configurations will involve either a floating ground, a single point ground, a multipoint ground, or some hybrid combination of these.
A floating ground configuration is illustrated in Figure 22. This type of signal ground system is electrically isolated from the ground and other conductive objects. Hence, noise currents present in the ground system will not be conductively coupled to the signal circuits. The floating ground system concept is also employed in equipment design to isolate signal returns from equipment cabinets and thus prevent unwanted currents in cabinets from coupling directly to signal circuits.

The effectiveness of floating ground systems depends on their true isolation from other nearby conductors; floating ground systems must really float. In large facilities, it is often difficult to achieve and maintain an effective floating system. Such a floating system is most practical if a few circuits or a few pieces of equipment are involved and power is applied from either batteries or dc-to-dc converters.
A single-point ground for an equipment complex is illustrated in Figure 23. With this configuration, the signal circuits are referenced to a single point, and this single point is then connected to the facility ground. The ideal single-point signal ground network is one in which separate ground conductors extend from one point on the facility ground to the return side of each of the numerous circuits located throughout a facility. This type of ground network requires an extremely large number of conductors and is not generally economically feasible. In lieu of the ideal, various degrees of approximation to single point grounding are employed.

The configuration illustrated in Figure 24 represents a ground bus arrangement that is often used to provide an approximation to the single-point grounding concept. The ground bus system illustrated in Figure 24 assumes the form of a tree. Within each system, the individual subsystems are single-point grounded. Each of the system ground points is then connected to the tree ground bus with a single insulated conductor.

The single-point ground accomplishes each of the three functions of signal circuit grounding. That is, a signal reference is established in each unit or piece of equipment, and these individual references are connected together. These, in turn, are connected to the facility ground at least at one point, which provides fault protection for the circuits and provides control over static charge buildup.
An important advantage of the single-point configuration is that it helps control conductively coupled interference. As illustrated in Figure 23, closed paths for noise currents in the signal ground network are avoided, and the interference currents, or voltages in the facility ground system, are not conductively coupled into the signal circuits via the signal ground network. Therefore, the single-point signal ground network minimizes the effects of any noise currents that may be flowing in the facility ground.
In a large installation, a major disadvantage of a single-point ground configuration is the requirement for long conductors. In addition to being expensive, long conductors prevent realization of a satisfactory reference for higher frequencies because of large self-impedances. Furthermore, because of stray capacitance between conductors, single point grounding essentially ceases to exist as the signal frequency is increased. In general, for typical equipments, systems, or facilities, single-point grounds tend to be optimum for frequencies below approximately 300 kHz.
The multiple-point ground illustrated in Figure 25 is the third configuration frequently used for signal ground networks. This configuration establishes many conductive paths to various electronic systems or subsystems within a facility. Within each subsystem, circuits and networks have multiple connections to this ground network. Thus, in a facility, numerous parallel paths exist between any two points in the multiple point ground network.
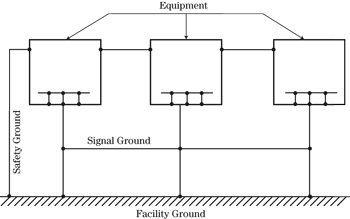
Multiple-point grounding frequently simplifies circuit construction inside complex equipment. It permits equipment employing coaxial cables to be interfaced more easily, since the outer conductor of the coaxial cable does not have to be floated relative to the equipment cabinet or enclosure.
However, multiple-point grounding suffers from an important disadvantage. Power currents and other high-amplitude, low-frequency currents flowing through the facility ground system can conductively couple into signal circuits to create intolerable interference in susceptible low-frequency circuits. Also, multiple ground loops are created, and this makes it more difficult to control radiated emission or susceptibility resulting from the common-mode ground loop effects. In addition, for multiple-point grounding to be effective, all ground conductors between the separate points must be less than 0.1 wavelength of the interference signal. Otherwise, common-ground impedance and ground radiated effects will become significant. In general, multiple-point grounding configurations tend to be optimum at higher frequencies (i.e., above 30 MHz).

To illustrate one form of a hybrid-ground system, Figure 26 shows a 19-in cabinet rack containing five separate sliding drawers. Each drawer contains a portion of the system (top to bottom): (1) RF and IF preamp circuitry for reception of microwave signals, (2) IF and video signal amplifiers, (3) display drivers, displays, and control circuits, (4) low-level audio circuits and recorders for documenting sensitive multichannel, hard-line telemetry sensor outputs, and (5) secondary and regulated power supplies. The hybrid aspect results from:
- The RF and IF video drawers are similar. Here, unit-level boxes or stages (interconnecting coaxial cables are grounded at both ends) are multipoint grounded to the drawer-chassis ground plane. The chassis is then grounded to the dagger pin, chassis ground bus as suggested in Figure 27. The power ground to these drawers, on the other hand, is using a single-point ground from its bus in a manner identical to the audio drawer.
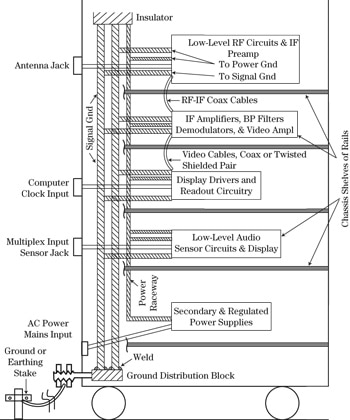
- The chassis or signal ground and power ground busses each constitute a multipoint grounding scheme to the drawer level. The individual ground busses are single-point grounded at the bottom ground distribution block. This avoids circulating common-mode current between chassis or signal ground and power grounds, since power ground current can vary due to transient surges in certain modes of equipment operation.
- Interconnecting cables between different drawer levels are run separately, and their shields, when used, are treated in the same grounding manner as at the drawer level.
- The audio and display drawers shown in Figure 27 use single-point grounding throughout for both their unit-level boxes (interconnecting twisted cable is grounded at one end to its unit) and power leads. Cable and unit shields are all grounded together at the common dagger pin bus. Similarly, the outgoing power leads and twisted returns are separately bonded on their dagger pin busses.
To review the above scheme, the following is observed:
- The audio and display drawers have ground runs of about 0.6 m and an upper frequency of operation of about 1 MHz (driver and sweep circuits). Thus, single-point grounding to the strike pins is indicated.
- The RF and IF drawers process UHF and 30 MHz signals over a distance of a meter so that multipoint grounding is indicated.
- The regulated power supplies furnish equipment units having transient surge demands. The longest length is about 1.5 m, and significant transient frequency components may extend up in the HF region. Here, hybrid grounding is indicated: single-point within a drawer and multipoint from the power bus to all drawers.
EMI Control Devices and Techniques
The performance of some EMI control techniques or devices may be significantly influenced by grounding. In particular, cable shields; isolation transformers; EMI filters; ESD, lightning, and EMF protection techniques; and Faraday shields must be properly grounded so as to provide maximum EMI protection. A detailed discussion of specific grounding considerations associated with these EMI control techniques or devices is beyond the scope of this book. However, it is important to emphasize the importance of grounding on the performance of these techniques or devices, and details may be found in the references.
Suggested Readings
[1] Morrison, Ralph, and W. H. Lewis, Grounding and Shielding in Facilities, Hoboken, NJ: John Wiley & Sons, 1990.
[2] Morrison, Ralph, Grounding and Shielding Techniques in Instrumentation, 3rd ed., Hoboken, NJ: John Wiley & Sons, 1990.
[3] Denny, Hugh W., Grounding for the Control of EMI, Gainesville, VA, Interference Control Technologies, Inc.
[4] Grounding, Bonding and Shielding for Electronic Equipment and Facilities, MIL-HDBK-419.