Numerical models can provide data from enormous numbers of points and for a number of human simulants
Alastair R. Ruddle, PH.D., MIRA Limited Nuneaton, UK
On-board transmitters are already commonly encountered in the vehicle environment; typical examples include mobile telephones, Bluetooth systems, and communications equipment used by the emergency services and armed forces. Wireless communication is also a key element of many schemes that aim to reduce the environmental impact of transport or to provide the pervasive network access that is envisaged in future urban environments, including vehicle passengers and their communication devices. Consequently, the use of on-board transmitters, both vehicle-mounted and personal, is likely to become more widespread in the future.
In recent years, various national and international bodies have recommended exposure limits for static electric and magnetic fields, as well as for electromagnetic fields at frequencies up to 300 GHz.[1-2] In Europe, the EU has recommended field exposure limits for the general public,[3] while occupational exposure limits are the subject of a specific directive.[4] Furthermore, the recently updatedEuropean Automotive EMC Directive[5] now requires vehicle manufacturers to provide guidelines relating to acceptable frequencies, power levels, and antenna installations to ensure that aftermarket equipment does not become a source of EMI problems. Perhaps such guidance should take account of occupant field exposure, as well as purely EMC issues.
Manufacturers of vehicles and mobile communications equipment, as well as potential users such as the emergency and armed services, should therefore take account of the possible human exposure implications of on-board transmissions. This article outlines some of the particular issues concerning in-vehicle field exposure assessment and summarizes specific findings from simulation-based studies carried out for vehicle-mounted and personal transmitters operating at TETRA frequencies (390- 430 MHz).
In-Vehicle Exposure Assessment
Electromagnetic field exposure guidelines are typically framed in terms of “reference levels” (or “action values”) and “basic restrictions” (or “exposure limit values”). The “reference levels” relate to quantities such as electric and magnetic field strengths, while the “basic restrictions” are specified in terms of quantities such as currents induced in the body, power density, and SAR (specific absorption rate). Some standards specify SAR as an average over 1 g of contiguous tissue, while in others a 10-g average is used. Limits are specified for whole body averages and for local maxima, which may vary between different parts of the body. Compliance with the reference levels is assumed to guarantee compliance with the basic restrictions in non-localized uniform exposures, but the use of reference levels is not considered to be appropriate for the highly localized sources presented by personal transmitters.
However, it is less clear how this approach translates to vehicles or buildings, where the local field may be significantly modified by reflections from the environment. In such resonant environments, the exposure caused by non-localized sources may be highly non-uniform and could be so complex that reliable assessment by measurements become difficult.
Statistical measures provide a more appropriate description of such field distributions, but this requires the availability of large numbers of samples. Simulation is therefore of increasing interest as a means of obtaining the large datasets needed to obtain reliable statistics without the limitations of physical tests. These include the chamber time needed for detailed mapping of field distributions, spurious field disturbances that may be introduced by field transducers and their positioning equipment, and the physical access restrictions that result from using probes of finite size in vehicles of complex geometry.
In both measurements and simulations, it is much easier to assess compliance with the reference levels than with the basic restrictions. Physical “phantoms” are required to represent the human body in measurements (as in McCoy et al.[6]), while “human simulants” need to be included in numerical models. Moreover, it may be necessary to consider the impact of different vehicle occupancy configurations, and the resulting need for several flexible whole-body phantoms could make measurement-based approaches a very expensive option. Numerical models, by contrast, can provide data from enormous numbers of points and for a number of human simulants at little additional cost.
A further benefit of simulation-based approaches is that investigations can be carried out during the early phases of development. The cost of any mitigation measures that might be found necessary is generally much lower if introduced at the design stage rather than in the later stages of the development lifecycle, when prototypes become available for measurements. Thus, simulation-based methods are of interest as potentially more comprehensive, more efficient, and more cost-effective than the measurement-based alternative.
Numerical Models
Comparative measurements up to 1 GHz[7] indicate that seat cushions and simple window glazing (and by implication, other interior trim materials) do not have a significant impact on the coupling of electric fields into the interior of a vehicle at these frequencies. Comparative simulations[8] show that window heater arrays modify the coupling between interior points and off-board antennas and are, therefore, expected to impact the internal field distribution.
Consequently, occupant field exposure evaluations up to 1 GHz require a model comprising the vehicle body-shell and doors, as well as the major metallic parts in the passenger compartment, such as seat frames, window heater arrays, and interior steering components. Simulations of such systems have been shown[9] to provide a good correlation with electric field mapping carried out on a complete vehicle at TETRA frequencies. At higher frequencies, however, it is likely that additional components, such as window glass, would also need to be included.
Simulations of derived quantities such as SAR require models of the human occupants to be added to the basic vehicle model. However, seating positions vary between vehicle types, and between vehicles of similar type, as well as between front and rear seats of the same vehicle. The latter is illustrated in Figure 1, which shows the relative geometry of three human simulants and the metallic elements of the seats of a car. Thus, it is necessary to be able to adjust the geometry of the human simulants to obtain suitable occupant models.
These variants make the definition of a “standard” human simulant for in-vehicle SAR calculations somewhat difficult. Localized distortions are likely, and features such as the volume may be slightly modified between models representing occupants in different seating positions. For these reasons, the use of homogenous lossy dielectric bodies with electrical properties that are representative of the average for human tissues can help to reduce the effort involved in adjusting the basic human simulant geometry. It is reported[11] that average permittivity and conductivity values for the body as a whole can be approximated as two-thirds of the values for human muscle tissue. Measured data for the latter are available.[12]
This homogenized simulation approach is similar to the experimental methods, which use phantoms based on dielectric shells filled with liquids with properties that are representative of human tissue. Nonetheless, this is not a fundamental limitation for simulations, and a model with an anatomically detailed model of a car driver has been demonstrated.[10]
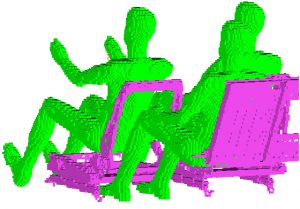

Roof-Mounted Tetra Antenna
A model representing a vehicle excited with a simple monopole antenna mounted at the rear of the roof panel is shown in Figure 2, illustrated with four homogenized human simulants inside. Simulations based on this system have been used to investigate the field distribution in the empty vehicle, as well as the SAR distributions for the eight possible combinations of a driver and up to three passengers. In this work the simulations were carried out using a commercial tool [13] based on the TLM (transmission line modeling) technique.[14]
In the simulations for TETRA frequencies (380-430 MHz), the discretization applied over the vehicle interior is such that electric and magnetic field data are computed at more than 1.8 million points over the passenger compartment, while SAR values are available for around 53,000 points per human simulant.
The field distributions obtained for the empty passenger compartment are such that the maximum field values are considerably higher (by two orders of magnitude in some cases) than the average for the interior as a whole. However, the highest field levels are generally found very close to metallic structures and are largely confined to areas that would not normally be accessed by the occupants. The most notable exception is the steering wheel.
For the particular geometry considered here, the average field strengths obtained from the empty vehicle model over the regions occupied by the human simulants are found to be at similar levels to the average field strengths over the passenger compartment as a whole. In this case, therefore, the average empty vehicle field strength appears to be a useful measure to describe the in-vehicle field exposure threat for occupants in typical seating locations.
Sample spatial electric field results derived from the empty and driver-only models are illustrated in Figures 3, 4 (with and without the driver, respectively, using the same scale), while Figure 5 shows the computed SAR for the driver (averaged over 10 g of contiguous tissue in this case, as specified in EC recommendations and requirements.[3, 4]). Differences can be seen between the field distributions of Figures 3, 4, which are caused by the presence of the driver. Overall the field strengths are generally lower with the driver present, but not always. In Figures 3, 4, for example, the field between the floor pan and rear seat back (see lower right-hand corner) is slightly enhanced with the driver present.
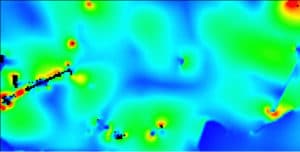
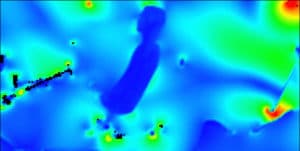
The occupied vehicle simulations[15] indicate that the average SAR limits are reached at lower radiated power levels than the maximum SAR limits, and that the highest peak and average SAR levels occur for different vehicle occupancy configurations. Although the antenna is located at the rear of the roof, the highest local SAR is found in the driver with a single passenger in the front, at almost double the driver only level for both the 1-gm and 10-gm SAR averages (although the mean SAR is only 70% of the driver-only levels). The maximum driver level is also 50% higher than driver-only levels for the three-occupant case where the two passengers are adjacent to the driver.
The highest mean SAR levels are found to occur in a single rear passenger – i.e., 25% higher than for the driver alone for the position behind the driver, and 50% higher for the position behind the front passenger seat. The distribution of the mean SAR values between the occupants is found to be very similar for both the 10-g and 1-g averages. Greater disparities are found for the maximum SAR values, but the highest levels are still found for the same locations and occupancy configurations.
In the case studied here, it is found that radiated power levels resulting in average empty vehicle field levels of 3.1 times the reference levels in the EC recommendation[3] would be needed to reach the average SAR limit for the driver alone. Taking account of the different occupancy configurations, the safety factor provided by the field reference levels is reduced slightly (from 3.1 to 2.5). Thus, assessing the average empty vehicle field distribution against the field reference levels still appears to be a reliable approach in this case.
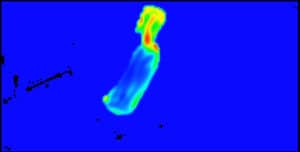
Personal Tetra Transmitter
The personal system that has been analyzed used a simplified representation of a personal radio system worn on the chest of the driver. This model employed a simple “zig-zag” monopole element to obtain the required resonance at a realistic physical length. The antenna was excited against a small conducting block representing the equipment housing. A TLM model for the vehicle occupied by the driver only is shown in Figure 6, illustrating the locations of the human simulant and the body-worn radio transmitter.
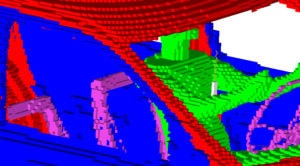
Simulations with Vehicle Structure
Simulations were again carried out for the unoccupied vehicle, and for models that included the driver and combinations of up to three passengers.[16] For the driver wearing the transmitter, maximum SAR is found to be the more immediate threat than mean SAR, as might be expected for a body-worn transmitter. Furthermore, it is found that the maximum SAR limits can be reached at power levels for which the mean fields over regions of the empty vehicle are only ~27% of the field reference levels recommended. [3] Thus, assessing compliance with the field reference levels is not an appropriate method for assessing exposure risks for the wearer of a body-worn antenna, as expected.
The results also suggest that the maximum and mean SAR levels for a passenger seated next to the driver, and for a rear passenger seated in the position behind the front passenger, are negligible (< 1.5%) compared to the levels resulting in the driver. However, the maximum SAR levels predicted for a rear passenger located behind the driver are as high as they are in the driver, although the mean SAR for this passenger is only around 65% of the mean levels for the driver. These trends are found to be independent of both the number and the disposition of other passengers within the vehicle.
In the case studied here, the highest SAR levels occur in the driver’s trunk, while for the passenger immediately behind the driver a similarly high SAR level is found in the limbs. The maximum SAR limits of the EC recommendation[3] are actually 2 W/kg for the head and trunk, but 4 W/kg for limbs. Thus, although the maximum SAR levels are the same in both of these simulants, the EC limits[3] would permit levels in the rear passenger that are twice those that would be acceptable in the driver. Even at the 4-W/kg limit, however, the projected mean fields over the passenger position behind the driver are still found to be below the field reference levels (at 53% for electric field and 50% for magnetic field). In this case, therefore, the field reference levels also appear to be inappropriate for assessing the exposure risk for at least one occupant other than the user.
Simulations without Vehicle Structure
Simulations with the vehicle structures removed from the models, such that the relative positions of the human simulants are unchanged, have also been used to assess the influence of the vehicle on the exposure. Although the 10-g SAR levels and the mean 1-g SAR for the driver alone are all slightly enhanced by the car, the 1-g maximum is slightly reduced. Nonetheless, the differences are found to be small (< 3%) in all cases. Thus, the very low SAR levels in the front and rear passengers to the side of the driver, as well as the rather higher and similar SAR levels found in the driver and in the passenger seated immediately behind the driver, appear to be dominated by the relative geometry of the human simulants and the transmitting antenna.
However, the details of the SAR distribution are modified by the presence of the vehicle structure. This is illustrated in Figures 7, 8, which show the computed SAR distribution in a vertical plane through the driver and adjacent rear passenger, with and without the vehicle.
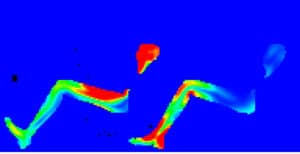
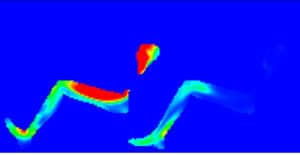
In the vehicle, the highest SAR levels in the passenger behind the driver occur in the ankles. With the vehicle structures removed, the highest SAR levels in the individual behind the user occur in the hand that is closest to the transmitter. The SAR distribution in the user is also modified by the vehicle structure, but less profoundly than for the rear passenger.
Conclusions
Simulation-based methods for assessing in-vehicle human field exposure risks caused by on-board transmitters offer a number of advantages over test-based methods. However, assessing compliance with basic restrictions, such as SAR, results in significant additional complications in both measurements and simulations. Thus, assessment methods based on comparing empty vehicle fields with reference levels are a much more desirable solution, provided that their use is justifiable.
Simulations representing a vehicle-mounted antenna and a personal transmitter worn by the driver of a passenger car have been carried out for eight different occupancy schemes involving a driver and up to three passengers for TETRA frequencies (380-430 MHz). Results obtained for the roof-mounted antenna suggest that mean SAR limits are a more immediate threat than peak SAR, but that mean SAR limits would not be breached unless the average empty vehicle fields reach 2.5 times the field reference levels.
For the driver-worn personal transmitter, the simulations confirm that peak SAR limits are a more immediate threat than mean SAR and that the reference levels are inappropriate for assessing the localized exposure experienced by the user. However, it is also found that the peak SAR level in a passenger seated immediately behind a driver wearing the transmitter may be as high as that for the driver. This situation is independent of whether other passenger locations are occupied, and even whether the vehicle structure itself is present. Thus, establishing compliance with the reference levels may not be a reliable approach for assessing the field exposure risks for individuals in close proximity to the user of a personal system.
The effects of the vehicle structure on the maximum and mean SAR levels, and their relative distribution amongst the human simulants, are relatively small for the personal transmitter. However, the vehicle structure does modify the details of the SAR levels and the distribution within the human simulants. Since the basic restrictions vary between parts of the body, SAR results obtained using human simulants without the vehicle structure cannot be relied upon to establish compliance with the SAR limits. This conclusion would apply not only to human simulants used in numerical models, but also to the physical phantoms used in measurements.
Although peak SAR limits are reached for average empty vehicle fields at ~27% of the reference levels for the personal transmitter studied here, systems that produce average empty vehicle fields that are considerably below the reference levels can probably be assumed to be compliant with the basic restrictions. Using 10% of the reference level as the evaluation criterion would provide a similar safety margin to that found for the roof-mounted antenna (i.e., a factor of ~2.5).
Further Work
Results from the roof-mounted antenna simulations suggest that comparing average empty vehicle field levels with field reference levels should provide a good safety margin in field exposure assessments for vehicle-mounted antennas. However, further investigation of other antenna locations and operating frequencies is required to establish whether the observations concerning the particular case studied here are more generally valid.
The personal transmitter simulations suggest that investigation of SAR levels for vehicle occupants can probably be avoided for personal systems operating at radiated power levels producing sufficiently low field strengths. However, investigation of a wider range of frequencies and source locations is needed to establish under what conditions this approach could be used with confidence.
Further investigation of the SAR levels and distributions results from other passengers as users of body-worn transmitters is also required to establish whether the wearer of a personal transmitter inside a vehicle is always subject to the highest field exposure levels and can, therefore, be assumed to represent the “worst-case” for analysis purposes.
A collaborative research project (SEFERE-“Simulation of Electromagnetic Field Exposure in Resonant Environments”) has recently been initiated in this area. This work is supported by the UK Government’s Department of Trade and Industry and aims to investigate field exposure simulation issues for a wider range of frequencies and applications than those described above. Results from this activity will be made available on the associated project website[17] as the work progresses.
References
- ICNIRP, “Guidelines for limiting exposure to time-varying electric, magnetic, and electromagnetic fields (up to 300 GHz)” Health Physics, Vol. 74, No. 4, 1998, pp. 494-522.
- C95.1-1999, “IEEE standard for safety levels with respect to human exposure to radio frequency electromagnetic fields, 3 kHz to 300 GHz” IEEE, 1999.
- 1999/519/EC, “Council Recommendation of 12th July 1999 on the limitation of exposure of the general public to electromagnetic fields (0 Hz to 300 GHz)”, Official Journal of the EC, No. L 199, 30th July 1999, pp. 59-70.
- 2004/40/EC, “Directive of the European Parliament and of the Council of 29th April 2004 on the minimum health and safety requirements regarding the exposure of workers to the risks arising from physical agents (electromagnetic fields)”, Official Journal of the EU, No. L 184, 24th May 2004, pp. 1-9.
- 2004/104/EC, “Commission Directive 2004/104/EC of 14th October 2004 adapting to technical progress Council Directive 72/245/EEC relating to the radio interference (electromagnetic compatibility) of vehicles and amending directive 70/156/EEC on the approximation of the laws of the Member States relating to the type-approval of motor vehicles and their trailers”, Official Journal of the EU, No. L 337, 13th November 2005, pp. 13-58.
- D.O. McCoy, D.M. Zakharia and Q. Balzano, “Field strengths and specific absorption rates in automotive environments”, IEEE Transactions on Vehicular Technology, Vol. 48, No. 4, July 1999, pp. 1287-1303.
- A.R. Ruddle, “Measured impact of seats and glazing on the coupling of electromagnetic fields into vehicles and their wiring harnesses”, Proceedings of 15th International Zurich EMC Symposium, Zurich, Switzerland, February 2003, pp. 487-492.
- A.R. Ruddle, “Computed impact of optional vehicle features (sunroof and windscreen heater) on automotive EMC characteristics”, Proceedings of 15th International Zurich EMC Symposium, Zurich, Switzerland, February 2003, pp. 475-480.
- A.R. Ruddle, “Comparison of measured and computed electric field distributions due to vehicle-mounted antennas”, Interference Technology EMC Test and Design Guide 2005, pp. 8-16.
- G. Anzaldi, E.C. Delgado, P.J. Riu, and F. Silva, “FDTD analysis of SAR from a cell phone inside a vehicle”, Proceedings of 16th International Zurich EMC Symposium, February 2005, pp. 155-160.
- C.H. Durney, H. Massoudi, and M.F. Iskander, “Radiofrequency radiation dosimetry handbook”, 4th Edition, Brooks Air Force Base Technical Report USAFSAM-TR-85-73, October 1986.
- C. Gabriel and S. Gabriel, “Compilation of the dielectric properties of body tissues at RF and microwave frequencies”, Brooks Air Force Base Technical Report AL/OE-TR-1996-0037, June 1996.
- D.P. Johns, R. Scaramuzza, and A.J. Wlodarczyk, “Micro-Stripes-microwave design tool based on 3D-TLM”, Proceedings of 1st International Workshop on Transmission Line Matrix (TLM) Modeling-Theory and Applications, Victoria, BC, Canada, August 1995, pp. 169-177
- C. Christopoulos, The Transmission-Line Modeling Method: TLM, IEEE Press, 1995.
- A.R. Ruddle, “Impact of passenger distribution on computed electromagnetic field exposure for vehicles with on-board transmitters”, Proceedings of EMC Europe Workshop on EMC of Wireless Systems, Rome, September 2005, pp. 411-414.
- A.R. Ruddle, “Influence of passengers on computed field exposure due to a personal TETRA radio used inside a vehicle”, Proceedings of 7th European EMC Symposium, Barcelona, Spain, September, 2006 pp. 592-597.
- SEFERE Project, see www.sefere.org.
Alastair Ruddle is with MIRA Ltd (Nuneaton, UK), where his work is largely concerned with the use of numerical modeling methods to investigate electromagnetic issues, primarily for automotive applications. Typical applications include EMC, installed performance of antennas, human exposure to electromagnetic fields, and associated test and measurement issues. He can be contacted at alastair.ruddle@mira.co.uk.