Imagine a radio frequency wavelength about the thickness of your pinky nail. This is a new area for technology applications.
A growing area in spectrum development is exploding. For many decades, very high frequencies (millimeter waves) have been the playground of radio-astronomers to image our solar system, stars, and galaxies. This space is “new” fertile ground for sensing and communications in terrestrial (and extra-terrestrial) use.
The millimeter wave space is opening up for these applications, which promise enormous multi-gigahertz bandwidths for innovation across many sectors.
Typically reserved for passive sensing of the mysteries of the Earth and our galactic environment, the 100+ GHz spectrum is, in a way, “off to the races.”
Radar applications for mobile devices (automobile and other) are being allocated on an increased basis. The applications are numerous, and the challenges are unique.
Because of the physics of dealing with these high frequencies, the use of these frequencies is confined to very focused applications, requiring precision and tight control on propagation between the devices that transmit and receive mmWave energy.
In the industry, the mmWave Coalition works on these new areas, monitoring and focusing on the allocations mandated by the Federal Communications Commission (FCC) and other regulatory bodies.
Tossed in the salad of interests are incumbent users of this spectrum (NASA, radio-astronomy users, research institutions as mentioned before) and a host of Earth- and space-based sensing systems.
What is tricky is the reasonable parsing of these new spectrum spaces. As terahertz (THz) applications are borne and deployed, there is a balance that must be considered for the use and protection of the incumbents without stifling innovation from other interests.
This is doubly necessary as the existing and future roles of terahertz technologies include Earth observations of weather and climate.
The radiometry applications (sensing at a distance based on the “temperature” of the Earth’s surface, as measured by wide-band sensors) are critical.
Additional clutter and noise in the GHz frequencies can clog and distort the science that is necessary to predict winds, waves, ocean, Earth’s surface temperature and other weather and physical phenomena that give eyes to what is happening on our planet.
Something as mundane as the nightly weather report is dependent on good satellite data for small and large weather patterns that affect daily life, crop predictions, safety, and incident prediction.
Other applications use radiometers to predict surface winds, all important for naval and ocean-going navigation and planning (see: Tropical Rainfall Measurement Mission).
As NASA explains: “TRMM provided much needed information on rainfall and its associated heat release that helps to power the global atmospheric circulation that shapes both weather and climate. In coordination with other satellites in NASA’s Earth Observing System.”
- Various groups are very interested in this particularly large chunk of the spectrum. For the present, the FCC’s allocations end at 90GHz and the radio frequency spectrum, challenged and fiercely protected by research and other groups, is being weighed as new applications arise.
- Members of the mmWave Coalition include several applications-based organizations that are learning to capitalize and expand on mmWave capabilities. One member, for instance, equipment manufacturer Virginia Diodes, can expand sensing and measurements and, with their extension modules can detect, measure and synthesize frequencies in excess of 1000 GHz, which is remarkable.
According to the commonly used equation, the wavelength of an RF signal is equal to c/f (speed of light divided by frequency), or 300*10^8/1000X10^9. This means that the wavelengths are on the order of 0.3 millimeters, or about the thickness of your pinky nail.
What is amazing is the high frequencies allow for multi-GHz wide bandwidths, which allow for tremendous gains in communication operating data rates.
With these very small wavelengths, the physical structure of antennas and associated waveguides are attendantly small (we are talking pin-head small), precisely machined and carefully aligned. Care is required for connecting waveguide structures — no dirt and grime and grit, or the game is off.
The real challenges arise in making and sensing these frequencies because a few hindrances need to be noted and properly dealt with, namely the directionality of these mmWaves and the propagation losses associated with these very high frequencies.
In addition, the beamwidths of these high frequency phenomena are extremely tight. No longer can a large and lopey broadband antenna be used to measure this energy properly. Laser-like precision is necessary to collect and quantify these quantities.
We have worked on a system that used a 275 GHz operating frequency to sense and image packages for safety and security measures. Automotive radar applications at ~60 GHz are now commonplace.
PROPAGATION LOSSES
The propagation losses are extremely high, which limits the practical range for these applications. The Free-Space Propagation Losses (FSPL) are well-known.
The FSPL are a function of frequency, fundamentally, and can be calculated according to the following equation:
FSPL = 20log(d) + 20 log(f) + 20log (4π/c) – GTx – GRx
Where d is the distance, in meters, f is frequency in Hertz and GTx and GRx are the transmit and receive gain of the antennas, respectively.
c is the speed of light in m/s and π is my favorite irrational constant.
All other terms aside, the driving function in the above equation is the frequency of the RF energy, so the loss monotonically increases with frequency. At 100 GHz, the losses are 40 dB higher than at 1 GHz, which is a significant increase in propagation loss.
But there are derived benefits from this naturally occurring FSPL, namely, there are isolation losses that occur as a result and, in addition, the tight beamwidths tend to allow more isolation between devices that may be sharing the same spectrum, thus, much of the communications and sensing applications are point-to-point and necessarily so.
There are naturally occurring losses in the atmosphere, due to water vapor absorption — and these losses are substantial (see the figure below, note the large losses at ~100 GHz and l85 GHz).
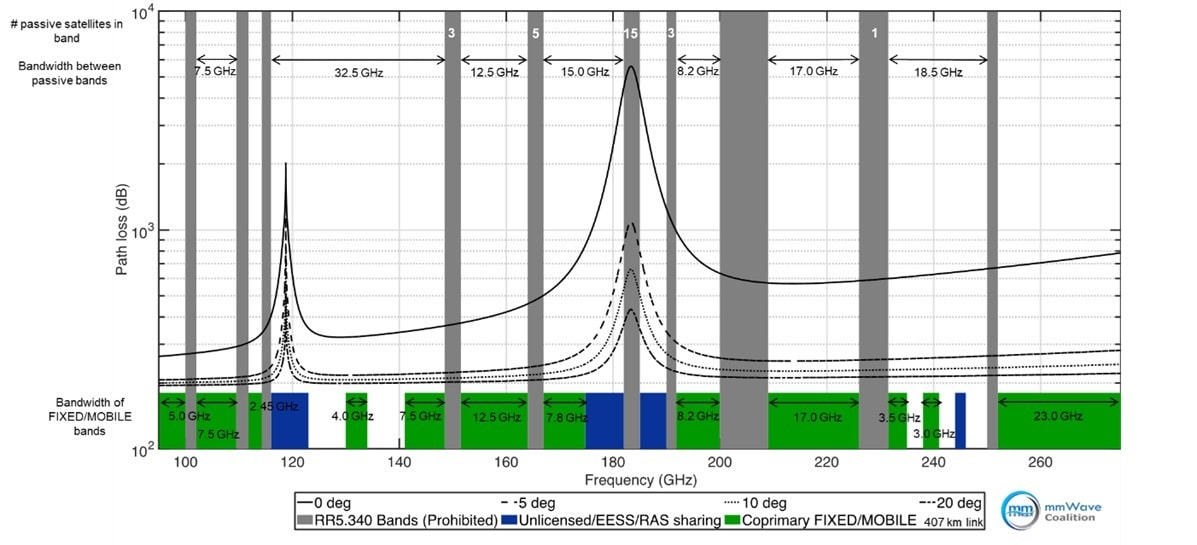
This is not at all a “bad” thing because one can take advantage of these deep losses to put sensitive communications in those bands.
For laboratory applications, it is critical that measurement personnel understand these physical realities and the correct use of measurement instruments be well-applied.
This demands that spectrum/receiver extension modules be correctly reasoned and applied. For example, most spectrum analyzer have baseband measurement input that can extend to 50GHz (or so).
Above that frequency extension modules use mixer-based extensions that “use” the local oscillator (LO) of the analyzer and beat the incoming signal to be measured against various harmonics of the LO.
This produces an input Intermediate Frequency (IF) that the analyzers can recognize and produce a display that, using conversion gains associated with the external mixer (from nominally 10 to 40 dB), can produce a calibrated response. Again, a little tricky, but not impossible.
As allocations increase, applications for mmWave are expanding. As the frequencies increase, the phenomena of RF communications begins to be more light-like and the cross-over between RF and optics becomes less hazy.
Innovative companies, such as those associated with the mmWave Coalition, are working to implement a balanced approach to use of these high frequency/short wavelength physics.
For the immediate future, the allocations for these devices are going to be negotiated and settled.
Stay tuned.